THE AARON KAPLAN LAB
Research topics
Research on the CO2 concentrating mechanism and related aspects:
Back in 1976 – as a post doc at the Carnegie Institution at Stanford we (together with Joe Berry and Murray Badger) discovered the CO2 concentrating mechanism in phytoplankton, first in the green alga Chlamydomonas reinhardtii and then in the cyanobacterium Anabaena variabilis (Kaplan, 2017). Together with Leonora Reinhold, Teruo Ogawa and later with Martin Hagemann, Wolfgang Hess and others, and many excellent students (listed as first authors) we made some of the major contributions to the field taking physiological, biochemical, cell cycle, genetic modification, gene regulation and modelling approaches. Together with Leonora Reinhold we uncovered the role of cyanobacterial carboxysomes, constructed the first carboxysomal models (Reinhold et al., 1986; Reinhold et al., 1989; Reinhold et al., 1991), and revealed the importance of proper organization of Rubisco therein (Schwarz et al., 1995). We uncovered the massive cycling of inorganic carbon between phytoplankton cells and their surroundings and its role in dissipating excess light energy (Tchernov et al., 1997; Tchernov et al., 2003). Recent studies related to the CCM focused on the function and regulation of photorespiration in cyanobacteria and its relation to the regulation of the CCM (Eisenhut et al., 2006; Eisenhut et al., 2008; Haimovich-Dayan et al., 2015; Orf et al., 2016). This also led to studies on the regulation between auto- and photo-mixotrophic metabolisms in cyanobacteria at the levels of physiological and gene expression responses (Kahlon et al., 2006; Haimovich-Dayan et al., 2011). Over the years, we wrote some of the main reviews/chapters in the field (Schwarz et al., 1993a; Schwarz et al., 1993b; Kaplan et al., 1994; Schwarz et al., 1994; Kaplan et al., 1997; Kaplan & Reinhold, 1999; Ogawa & Kaplan, 2002; Ogawa & Kaplan, 2003; Fukuzawa et al., 2012; Burnap et al., 2015, among others).
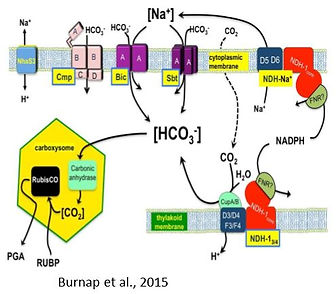
Regulation of photosynthetic electron transport in response to ambient conditions:
As the photosynthetic machinery “counts” the electrons leaving the water splitting system and those utilized in CO2 fixation, I became interested in the photosynthetic electron transport routes and their regulation. Early studies (Hassidim et al., 1997) focused on the regulation of the effective light harvesting cross section in a cyanobacterium as affected by the light intensity during growth and experimental conditions. As we were missing a route of electron dissipations downstream of PSII, we uncovered the flv1-4 genes and the FLV proteins involved in light dependent O2 uptake (Helman et al., 2003; Helman et al., 2005). We also uncovered an yet unexplained process of O2 uptake within PSII (Eisenstadt et al., 2010). Later experiments focused on the non-classical NPQ observed in what we often term “real life organisms” such as those inhabiting biological soil crusts (BSC, see below), oceanic diatoms and others. Here, unlike in model “laboratory pets”, chlorophyll fluorescence quenching mostly reflects events in reaction centers rather than in light harvesting complexes. The first studies were on the diatom Phaeodactylum tricornutum (Eisenstadt et al., 2008). This was followed by work performed with Itzhak Ohad and Nir Keren (Ohad et al., 2010) and recently on Chlorella ohadii (Treves et al., 2016), see below. Our studies on the photosynthetic ET revealed the resilience of the system to photodamage in these, as compared with model organisms.
The “languages” used by organisms in intra- and inter-species communication:
These studies were initiated in 1997 when we were following the collapse of a natural dinoflagellate, Peridinium gatunense, bloom in Lake Kinneret. We showed that the termination of the bloom is mediated by programmed cell death (PCD), which is activated by an oxidative stress developing due to CO2 limitation. We were among the first to show a role for PCD in a unicellular eukaryotic population and to provide a mechanistic explanation to: 1. the biological dimension to the evolutionary benefit of patchy growth habit (better defense against toxic cyanobacterial blooms, see below); 2. the apparent paradox that older phytoplankton cells are far more sensitive to oxidative stress than younger cells despite the fact that they possess far higher antioxidant activities; 3. the fact that the entire population collapse due to oxidative stress at the end of the bloom, of old and young cells alike, is mediated by a protease excreted by older cells sensitizing younger cells to oxidative stress (Vardi et al., 1999; Vardi et al., 2007). Among other aspects, these studies questioned the widely accepted dogma that cells that acquired detoxification of oxidant activities are better suited to cope with oxidative stress. In a series of studies we showed that this is clearly not the case. Peridinium and Chlamydomonas (used as a better model) cells that experienced oxidative stress were far more vulnerable to an additional H2O2 treatment than naïve cells. We then uncovered the mechanism involved. During the first stress they upregulate ascorbate peroxidase level and activity. Once they experience a second stress they produce dehydroascorbate (DHA), an intermediate in the ascorbate cycle, which serve as a surveillance metabolite “reporting” to the cells that they have already experienced such stress. Addition of DHA to naïve cells led to massive death via a classical PCD (Murik & Kaplan, 2009; Murik et al., 2014). We proposed that the evolutionary driving force for the altruistic cell death may be aimed at eliminating damaged cells (by the earlier stress) and thereby enhancing population fitness.
​
​
​
​
​
​
​
​
​
​
​
​
​
​
​
The biological role of secondary metabolites, some toxic:
The observation that toxic Microcystis sp. is present in Lake Kinneret but not in the Peridinium patches prompted us to initiate studies on the interspecies interactions and the biological role of secondary metabolites including cyanobacterial toxins (Vardi et al., 2002; Schatz et al., 2005; Schatz et al., 2007). Our studies (in cooperation with Elke Dittmann) unraveled two roles for microcystin, a protein phosphatase inhibitor (and thus toxic to eukaryotes): an internal and an external role. Inside the Microcystis cells it binds to specific proteins such as Rubisco and protects them against oxidative stress such as high illumination (Zilliges et al., 2011). When released to the medium by lysing Microcystis cells (due to various stresses) microcystin’s presence is sensed by neighboring cells. They respond by upregulating genes involved in stress reactions. Thus microcystins act as infochemicals reporting that the Microcystis population is under stress (Schatz et al., 2007; Kaplan et al., 2012). Ongoing studies on this topic and identification of secondary metabolites involved in Microcystis-bacterial interactions are described below.

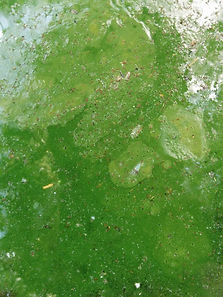
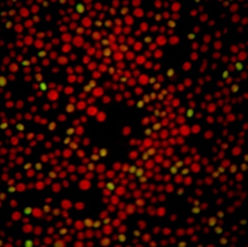
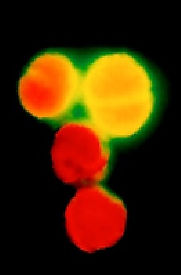
Studies on diatoms:
In addition to the photosynthetic ET and reaction centers, we took an important part in the analysis of a diatom (Phaeodactylum tricornutum) genome (Bowler et al., 2008) and that of its complex multi-compartmental carbon metabolism (Kroth et al., 2008). By silencing an essential gene of the C4 metabolism we showed that it does not fix Carbon through a C4 route, and that the latter is likely an additional means to dissipate excess light energy (Haimovich-Dayan et al., 2013).
Acclimation of biological soil crust (BSC)-inhabiting organisms:
On the premise that unique capabilities may be found in organisms experiencing extreme conditions, we focused on the adaptation of photosynthetic microorganisms in desert BSC, one of the harshest environments on Earth. Studies on cyanobacteria: Our analysis clarified the distribution of cyanobacteria and other bacteria along a South-to-North rainfall gradient (Hagemann et al., 2015; Hagemann et al., 2016). We mostly focused on the acclimation and responses to the hydration/desiccation cycles - early morning dew deposition followed by dehydration that the organisms face about 200 days a year. First we examined the mechanism whereby Microcoleus sp. is able to cope with the rising excess illumination, particularly when the photochemical activity declines during dehydration. As mentioned, they activate a non-radiative recombination in PSII minimizing the formation of damaging 1O2 (Ohad et al., 2010; Ohad et al., 2011). Field and laboratory studies indicated the complexity of the BSC and revealed that in fact most of the photosynthetic activity occurs beneath the surface where the light intensity is significantly damped. In that, the cyanobacteria population may be regarded as “low light” organisms, but able to withstand high illumination (Raanan et al., 2016a).
To clarify the cyanobacterial acclimation to the BSC conditions we constructed an environmental chamber whereby we can accurately and reproducibly simulate the conditions in the BSC at a given day using local meteorological data (Raanan et al., 2016b). We then examined physiological parameters, focusing on photosynthetic parameters that revealed large differences between dehydration-tolerant and sensitive cyanobacteria (Raanan et al., 2016c). Further, we sequenced Leptolyngbya ohadii isolated from the BSC (kept in axenic cultures, available on demand). Comparative sequencing identified a set of genes present in dehydration-tolerant but not in -sensitive cyanobacteria (Murik et al., 2017). Physiological experiments showed that the cells must prepare themselves to the forthcoming, inevitable, dehydration otherwise they are unable to revive thereafter. Use of RNAseq on RNA samples withdrawn during the a simulated dehydration profile indicated that of the 7487 genes recognized in Leptolyngbya, 4 h of dehydration caused the up-regulation in transcript level of 567 and down-regulation of 1597 by more than 2-fold. Recent studies implicated the rising dawn illumination as the signal sensed by the cells and identified the mechanism whereby the transcript profile is regulated.
​
​
​
​
​
​
​
​
​
​
​
​
Ongoing studies on a green algae:
Together with the late Professor Itzhak Ohad, we isolated a green alga from a BSC and I named it Chlorella ohadii (Treves et al., 2013). Perhaps related to the high illumination in its habitat, we found it completely resistant to photoinhibition and clarified the physiological mechanism involved (Treves et al., 2016; Ananyev et al., 2017). We then used a metabolome approach to gain a better understanding of its multiphasic unparalleled growth rate showing, among other aspects, the involvement of polyamines (Treves et al., 2017). Through a detailed sequence analysis and transcript profiling under various conditions and development of genetic manipulation capabilities, we now possess the essential information/ability to clarify what rate limits growth of photosynthetic organisms under what we may consider optimal conditions.
​
​
​
​
​
​
​
​
Biotechnological applications
with all due modesty we were the first to show significant improvement of the yield in model higher plants (Arabidopsis and tobacco), where we introduced a cyanobacterial (ictB) gene (Lieman-Hurwitz et al., 2003). This was later confirmed using several crop plants, the most recent one showed a large (23%) rise in the yield of Soybean expressing ictB (Hay et al., 2017). Currently we are planning to use C. ohadii genes to improve the growth of crop plants and to express foreign genes for high value products in C. ohadii.
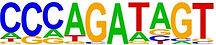
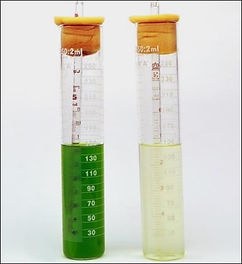

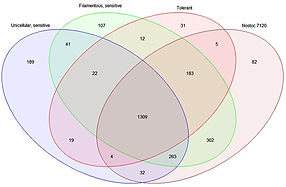
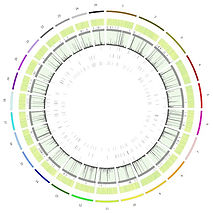
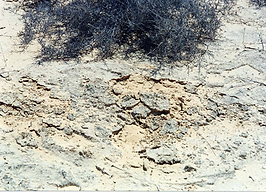
Current research is provided in the Lab members section
References
​
Ananyev F, Gates C, Kaplan A, Dismukes GC. 2017. Photosystem II-cyclic electron flow powers exceptional photoprotection and record growth in the microalga Chlorella ohadii Biochem Biophys Acta- Bioenergy in press.
Bowler C, Allen AE, Badger JH, Grimwood J, Jabbari K, Kuo A, Maheswari U, Martens C, Maumus F, Otillar RP, et al. 2008. The Phaeodactylum genome reveals the evolutionary history of diatom genomes. Nature 456: 239-244.
Burnap RL, Hagemann M, Kaplan A. 2015. Regulation of the CO2 concentrating mechanism in cyanobacteria. Life 5: 348-371.
Eisenhut M, Kahlon S, Hasse D, Ewald R, Lieman-Hurwitz J, Ogawa T, Ruth W, Bauwe H, Kaplan A, Hagemann M. 2006. The plant-like C2 glycolate cycle and the bacterial-like glycerate pathway cooperate in phosphoglycolate metabolism in cyanobacteria. Plant Physiol 142: 333-342.
Eisenhut M, Ruth W, Haimovich M, Bauwe H, Kaplan A, Hagemann M. 2008. The photorespiratory glycolate metabolism is essential for cyanobacteria and might have been conveyed endosymbiontically to plants. Proc. Natl. Acad. Sci. USA 105: 17199-17204.
Eisenstadt D, Barkan E, Luz B, Kaplan A. 2010. Enrichment of oxygen heavy isotopes during photosynthesis in phytoplankton. Photosynth. Res. 103: 97-103.
Eisenstadt D, Ohad I, Keren N, Kaplan A. 2008. Changes in the photosynthetic reaction center II in the diatom Phaeodactylum tricornutum result in non-photochemical fluorescence quenching. Environ Microbiol 10: 1997-2007.
Fukuzawa H, Ogawa T, Kaplan A 2012. The Uptake of CO2 by Cyanobacteria and Microalgae. In: Eaton Rye JJ, Tripathy BC, Sharkey TD eds. Photosynthesis: Plastid Biology, Energy Conversion and Carbon Assimilation: Springer, 625-650.
Hagemann M, Henneberg M, Felde V, Berkowicz S, Raanan H, Pade N, Felix-Henningsen P, Kaplan A. 2016. Cyanobacterial populations in biological soil crusts of the northwest Negev desert, Israel - effects of local conditions and disturbance. FEMS Microbiol Ecol (in press).
Hagemann M, Henneberg M, Felde VJMNL, Drahorad SL, Berkowicz SM, Felix-Henningsen P, Kaplan A. 2015. Cyanobacterial diversity in biological soil crusts along a rainfall gradient of the North/West Negev desert, Israel. Mol Ecol 70: 219-230.
Haimovich-Dayan M, Garfinkel N, Ewe D, Marcus Y, Gruber A, Wagner H, G. KP, Kaplan A. 2013. The role of C4 metabolism in the marine diatom Phaeodactylum tricornutum. . New Phytol. 197: 177-185.
Haimovich-Dayan M, Kahlon S, Hihara Y, Hagemann M, Ogawa T, Ohad I, Lieman-Hurwitz J, Kaplan A. 2011. Cross-talk between photomixotrophic growth and CO2-concentrating mechanism in Synechocystis sp strain PCC 6803. Environ Microbiol 13: 1767-1777.
Haimovich-Dayan M, Lieman-Hurwitz J, Orf I, Hagemann M, Kaplan A. 2015. Does 2-phosphoglycolate serve as an internal signal molecule of inorganic carbon deprivation in the cyanobacterium Synechocystis sp. PCC 6803? Environ Microbiol 17: 1794-1804.
Hassidim M, Keren N, Ohad I, Reinhold L, Kaplan A. 1997. Acclimation of Synechococcus strain WH7803 to ambient CO2 concentration and to elevated light intensity. J. Phycol. 33(5): 811-817.
Hay WT, Bihmidine S, Mutlu N, Hoang KL, Awada T, Weeks DP, Clemente T, Long SP. 2017. Enhancing soybean photosynthetic CO2 assimilation using a cyanobacterial membrane protein, ictB. J Plant Physiol http://dx.doi.org/10.1016/j.jplph.2017.02.003
Helman Y, Barkan E, Eisenstadt D, Luz B, Kaplan A. 2005. Fractionation of the three stable oxygen isotopes by oxygen-producing and oxygen-consuming reactions in photosynthetic organisms. Plant Physiol 138: 2292-2298.
Helman Y, Tchernov D, Reinhold L, Shibata M, Ogawa T, Schwarz R, Ohad I, Kaplan A. 2003. Genes encoding A-type flavoproteins are essential for photoreduction of O2 in cyanobacteria. Curr Biol 13: 230-235.
Kahlon S, Beeri K, Ohkawa H, Hihara Y, Murik O, Suzuki I, Ogawa T, Kaplan A. 2006. A putative sensor kinase, Hik31, is involved in the response of Synechocystis sp. strain PCC 6803 to the presence of glucose. Microbiology-Sgm 152: 647-655.
Kaplan A. 2017. On the cradle of CCM research: discovery, development, and challenges ahead J Exp Bot DOI: https://doi.org/10.1093/jxb/erx122.
Kaplan A, Harel M, Kaplan-Levy RN, Hadas O, Sukenik A, Dittmann E. 2012. The languages spoken in the water body (or the biological role of cyanobacterial toxins). Frontier in aquatic Microbiology 3:138. doi: 10.3389/fmicb.2012.00138.
Kaplan A, Reinhold L. 1999. The CO2 concentrating mechanisms in photosynthetic microorganisms. Annu. Rev. Plant Physiol. Plant Mol. Biol. 50: 539-570.
Kaplan A, Ronen-Tarazi M, Tchernov D, Bonfil DJ, Zer H, Schatz D, Vardi A, Hassidim M, Reinhold L 1997. The inorganic carbon-concentrating mechanism of cyanobacteria: genes and ecological significance.In Peschek GA, Loeffelhardt W, Schmetterer G. The photosynthetic prokaryotes: Plenum Press, New York.
Kaplan A, Schwarz R, Lieman-Hurwitz J, Ronen-Tarazi M, Reinhold L 1994. Physiological and molecular studies on the response of cyanobacteria to changes in the ambient inorganic carbon concentration. In: Bryant D ed. The Molecular Biology of the Cyanobacteria. Dordrecht, The Netherlands: Kluwer Academic Pub., 469-485.
Kroth PG, Chiovitti A, Martin-Jezequel V, Mock T, Schnitzler-Parker M, Stanley MS, Kaplan A, Caron L, Gruber A, Weber T, et al. 2008. A model of the carbohydrate metabolism in the diatom Phaeodactylum tricornutum deduced from whole genome analysis. Plos One 3: e1426. doi:1410.1371/journal.pone.0001426.
Lieman-Hurwitz J, Rachmilevitch S, Mittler R, Y. M, Kaplan A. 2003. Enhanced photosynthesis and growth of transgenic plants that express ictB, a gene involved in HCO3- accumulation in cyanobacteria. Plant Biotechnol J. 1: 43-50.
Murik O, Elboher A, Kaplan A. 2014. Dehydroascorbate: a possible surveillance molecule of oxidative stress and programmed cell death in the green alga Chlamydomonas reinhardtii. New Phytol 202: 471-484.
Murik O, Kaplan A. 2009. Paradoxically, prior acquisition of antioxidant activity enhances oxidative stress-induced cell death. Environ. Microbiol. 11: 2301-2309.
Murik O, Oren N, Shotland Y, Raanan H, Treves H, Kedem I, Keren N, Hagemann M, Pade N, Kaplan A. 2017. What distinguishes cyanobacteria able to revive after desiccation from those that cannot: The genome aspect. Environ Microbiol 19: 535–550.
Ogawa T, Kaplan A. 2002. Inorganic carbon acquisition systems in cyanobacteria. . Photosynth Res 77: 105-115.
Ogawa T, Kaplan A. 2003. Inorganic carbon acquisition systems in cyanobacteria. Photosynth. Res. 77: 105-115.
Ohad I, Berg A, Berkowicz SM, Kaplan A, Keren N. 2011. Photoinactivation of photosystem II: is there more than one way to skin a cat? Physiol Plant 142: 79-86.
Ohad I, Raanan H, Keren N, Tchernov D, Kaplan A. 2010. Light-Induced changes within photosystem II protects Microcoleus sp. in biological desert sand crusts against excess light. Plos One 5: Journal.pone.0011000 e0011000
Orf I, Schwarz D, Kaplan A, Kopka J, Hess WR, Hagemann M, Klähn S. 2016. CyAbrB2 contributes to the transcriptional regulation of low CO2 acclimation in Synechocystis sp. PCC 6803. Plant Cell Physiol 57 2232-2243.
Raanan H, Felde VJMNL, Peth S, Drahorad S, Ionescu D, Eshkol G, Treves H, Felix-Henningsen P, Berkowicz SM, Keren N, et al. 2016a. The 3D structure and cyanobacterial activity in a desert biological soil crust. Environ Microbiol 18: 372-383.
Raanan H, Oren N, Treves H, Berkowicz SM, Hagemann M, Padec N, Keren N, Kaplan A. 2016b. Simulated soil crust conditions in a chamber system provide new insights on cyanobacterial acclimation to desiccation Environ Microbiol 18 414-426.
Raanan H, Oren N, Treves H, Keren N, Ohad I, Berkowicz SM, Hagemann M, Koch M, Shotland Y, Kaplan A. 2016c. Towards clarifying what distinguishes cyanobacteria able to resurrect after desiccation from those that cannot: The photosynthetic aspect. Biochim Biophy Acta-Bioenerg 1857: 715-722.
Reinhold L, Kosloff R, Kaplan A. 1991. A model for inorganic carbon fluxes and photosynthesis in cyanobacterial carboxysomes. Can J Bot 69: 984-988.
Reinhold L, Zviman M, Kaplan A 1986. Inorganic carbon fluxes and photosynthesis in cyanobacteria - a quantitative model.In Biggins J. Progress in photosynthesis research. Rhode Island , USA: Martinus Nijhoff Pub. 289-296.
Reinhold L, Zviman M, Kaplan A. 1989. A quantitative model for inorganic carbon fluxes and photosynthesis in cyanobacteria. Plant Physiol and Biochem 27: 945-954.
Schatz D, Keren Y, Hadas O, Carmeli S, Sukenik A, Kaplan A. 2005. Ecological implications of the emergence of non-toxic subcultures from toxic Microcystis strains. Environ Microbiol 7: 798-805.
Schatz D, Keren Y, Vardi A, Sukenik A, Carmeli S, Boerner T, Dittmann E, Kaplan A. 2007. Towards clarification of the biological role of microcystins, a family of cyanobacterial toxins. Environ Microbiol 9: 965-970.
Schwarz R, Lieman-Hurwitz J, Marco E, Ronen-Tarazi M, Ohad N, Hassidim M, C. G, Reinhold L, Kaplan A 1993a. The CO2-concentrating mechanism of cyanobacteria: elucidation with the aid of high-CO2-requiring mutants.In Murata N. Research in Photosynthesis, Kluwer Academic Pub. 437-440.
Schwarz R, Lieman-Hurwitz J, Marco E, Ronen-Tarazi M, Ohad N, Hassidim M, Gabai C, Kaplan A 1993b. Ecological implications of the CO2-concentrating mechanism of cyanobacteria.In Guerrero R, Pedros-Alio C. Trends in microbial ecology.: Spanish Society of Microbiology. 92-97.
Schwarz R, Lieman-Hurwitz J, Ronen-Tarazi M, Gabai C, Hassidim M, Reinhold L, Kaplan A 1994. The Fluxes of Inorganic Carbon and CO2-Dependent Genes Involved in the Cyanobacterial Inorganic Carbon-Concentrating Mechanism: A View on Some of the Open Questions.In Stal LJ, Caumette P. Microbial Mats: Structure, development and environmental significance: Springer-Verlag. 299-304.
Schwarz R, Reinhold L, Kaplan A. 1995. Low activation state of ribulose 1,5-bisphosphate carboxylase/oxygenase in carboxysome-defective Synechococcus mutants. Plant Physiol 108: 183-190.
Tchernov D, Hassidim M, Luz B, Sukenik A, Reinhold L, Kaplan A. 1997. Sustained net CO2 evolution during photosynthesis by marine microorganisms. Curr Biol 7: 723-728.
Tchernov D, Silverman J, Luz B, Reinhold L, Kaplan A. 2003. Massive light-dependent cycling of inorganic carbon between photosynthetic microorganisms and their surroundings. Photosynth Res 77: 95-103.
Treves H, Murik O, Kedem I, Eisenstadt D, Meir S, Rogachev I, Szymanski J, Keren N, Orf I, Tiburcio AF, et al. 2017. Metabolic flexibility underpins growth capabilities of the fastest growing alga. Curr Biol in press.
Treves H, Raanan H, Finkel MO, Berkowicz SM, Keren N, Kaplan A. 2013. A newly isolated Chlorella sp. from desert sand crusts exhibits a unique resistance to excess light intensity. FEMS Microbiol Ecol 86: 373-380
Treves H, Raanan H, Kedem I, Murik O, Keren N, Zer H, Berkowicz SM, Giordano M, Norici A, Shotland Y, et al. 2016. The mechanisms whereby the green alga Chlorella ohadii, isolated from desert soil crust, exhibits unparalleled photodamage resistance. New Phytol 210: 1229-1243.
Vardi A, Berman-Frank I, Rozenberg T, Hadas O, Kaplan A, Levine A. 1999. Programmed cell death of the dinoflagellate Peridinium gatunense is mediated by CO2 limitation and oxidative stress. Curr Biol 9: 1061-1064.
Vardi A, Eisenstadt D, Murik O, Berman-Frank I, Zohary T, Levine A, Kaplan A. 2007. Synchronization of cell death in a dinoflagellate population is mediated by an excreted thiol protease. Environ. Microbiol. 9(2): 360-369.
Vardi A, Schatz D, Beeri K, Motro U, Sukenik A, Levine A, Kaplan A. 2002. Dinoflagellate-cyanobacterium communication may determine the composition of phytoplankton assemblage in a mesotrophic lake. Curr Biol 12: 1767-1772.
Zilliges Y, Kehr JC, Meissner S, Ishida K, Mikkat S, Hagemann M, Kaplan A, Borner T, Dittmann E. 2011. The cyanobacterial hepatotoxin microcystin binds to proteins and increases the fitness of Microcystis under oxidative stress conditions. Plos One 6(3): e17615